OVERVIEW
Our lab studies how the brain monitors changes in body
temperature (via thermosensitive neurons), blood sodium (via
sodium-sensitive neurons)
and extracellular fluid osmolality (via
osmosensory neurons). Our goal is to define how these neurons
convert (transduce) these physicochemical parameters into electrical
signals that can be transmitted to relevant parts of the brain
(homeostatic osmoregulatory networks). We also study how the brain's
biological clock
(the suprachiasmatic nucleus) regulates the body's hydration status.
Specifically, we are interested in how clock neurons adapt the function
of osmoregulatory networks to changing conditions imposed by the
sleep-wake cycle.
Many of these issues are studied in the context of diseases that feature
osmoregulatory defects in the etiology of pathological symptoms; such as
dehydration, salt-dependent hypertension, sepsis and brain trauma. We
hope that our findings will lead to novel therapeutic treatments for
such conditions(see the
outcomes page).
BACKGROUND
Role of the hypothalamus in health and disease. The
hypothalamus (highlighted in blue) is a small but vital part of the
brain that regulates basic physiological parameters such as body
temperature, blood pressure, body weight and plasma osmolality (a
measure of the total solute concentration in blood). It also regulates
sex and reproduction, and it coordinates our body's response to stress.
The hypothalamus also encloses a master biological clock
(suprachiasmatic nucleus) that coordinates sleep and most circadian
rhythms.
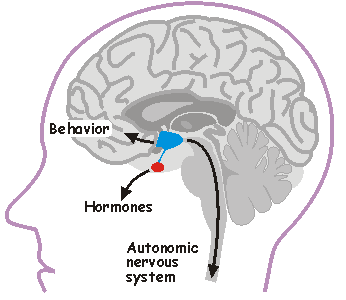
Hypothalamic neurons regulate body functions by affecting behavior via
projections to other parts of the brain (e.g. frontal cortex), by
commanding hormone release from the pituitary gland (red), through
descending neural projections (via the spinal cord) that regulate
autonomic output.
In the Bourque Lab, we are interested in how specialized hypothalamic
neurons detect changes in core body temperature, blood sodium
concentration and plasma osmolality. We are also interested in how these
central sensory neurons change the electrical activity of other
hypothalamic integrator, relay and effector neurons that regulate thirst
and release of the antidiuretic hormone vasopressin.
In healthy individuals, blood osmolality rises in proportion with the
concentration of sodium ions in blood, which changes as we loose or gain
water and salt. Gains in water and salt are due mainly to intake.
Because sweat is a hypotonic fluid, fluid lost for the purpose of
thermoregulatory evaporation causes mainly a water deficit. Water and
salt loss through urine production is under the control of hormones and
neurogenic influences. Osmolality and sodium concentration can also
increase when the concentration of other molecules rises during certain
disease states (e.g. high glucose levels in the absence of insulin). The
hypothalamus normally prevents large changes in osmolality through
systemic osmoregulation.
Systemic Osmoregulation in Health and Disease.
Systemic osmoregulation is the process by which our body maintains blood
osmolality near a value of 300 mosm/kg. Increases in in blood osmolality
(the ratio of salt to water) occur when we consume salt, when sweating
is triggered as a thermoregulatory response to hyperthermisa (e.g.
during exercise), or when we loose water through vomiting or
diarrhea. Blood osmolality drops when we ingest hypotonic fluids. From a
practical point of view, ingesting a tall glass of water or a few
packets of salt (like those in fast-food restaurants) is sufficient to
cause small but measurable changes in blood osmolality (within a few
minutes). Consuming larger amounts cause greater changes. Large changes
in osmolality are very dangerous because they can damage our cells and
organs by causing them to shrink or swell. The brain is particularly
sensitive, because it is encased in a rigid cranium. Changes in blood
osmolality near 10 mosmol/kg can simply cause headaches or irritability,
but larger changes can cause mental confusion, seizures, coma or even
death. A few years ago a person died after voluntarily ingesting a large
quantity of water (>2L) as part of a contest organized by a radio
station in California. Marathon runners also risk hypoosmolality
(dilutional hyponatremia) caused by excessive hydration. Young children
and the elderly are particularly vulnerable to hyperosmolality caused by
dehydration.
Hypothalamic control of osmoregulation. Systemic
osmoregulation depends on the existence of neurons capable of sensing
physiologically relevant changes in core body temperature
(thermoreceptors), extracellular sodium concentration (sodium detectors)
and fluid osmolality (osmoreceptors). These neurons, located in the
hypothalamus, transduce relevant stimuli into changes in electrical
(action potential) activity. This coded electrical message is then
transmitted, via synaptic contacts, to target neurons that integrate
different kinds of information so that the correct homeostatic response
can be generated. Osmoreceptors, sodium detectors and thermosensory
neurons control ingestive behavior by affecting the sensations of thirst
and salt appetite. They also regulate descending autonomic neurons that
control sympathetic outflow (to regulate blood pressure and renal
function), as well as neurons that release hormones to regulate salt and
water excretion at the kidney.
CURRENT RESEARCH TOPICS
Osmosensory transduction. We use various techniques to
define the cellular and molecular mechanisms by which osmoreceptors
detect changes in extracellular fluid osmolality. Our past work has
shown that osmosensory transduction involves mechanosensitive
(stretch-inhibited)
channels
that cause osmoreceptor neurons to depolarize under hypertonic
conditions (See more about this in the "patch-clamp" part of the
Techniques page). More recent studies in
our lab have shown that the gene coding for the capsaicin (hot pepper)
receptor, known as trpv1, contributes to the formation of this
channel and that an intact actin cytoskeleton is required for mechanical
control of the channel during osmosensory transduction. Current
projects aim to increase our understanding of the role of trpv1 (and
other genes) in osmosensory transduction. We are also interested in the
subcellular distribution and molecular architecture of the osmosensory
complex.
Glia-Neuron
signals and osmosensing. Glial cells contain and release the
amino acid taurine during hypotonicity. Taurine is an agonist of
inhibitory glycine receptors. We are therefore interested in the role
of glia, and glia-derived substances, in the osmotic control of neurons
involved in osmoregulation. In the supraoptic nucleus for example, the
somata of glial cells form a tight ventral glial lamina (VGL; purple
band)from where ascending astrocytitic processes intercalate between
vasopressin releasing neurons.
Thermosensory transduction. Ion channels encoded by
subtypes of transient receptor potential vanilloid (trpv) genes are
activated by heat in the near-physiological range. Our discovery that
trpv1 is expressed in neurons of the supraoptic nucleus led to the
hypothesis that these neurons might be thermosensitive. A study
published by Reza Sharif-Naeini from the Bourque Lab provided a first
indication that these neurons are intrinsically sensitive to changes in
temperature. Additional work is being performed to evaluate the
contribution of this response during physiological thermosensory
transduction.
Synaptic signals in osmoregutatory networks. Information
concerning core physiological parameters is encoded by
osmoreceptors, sodium detectors and thercoreceptors in the form of
changes in action potential firing. We are interested in the
mechanisms by this information is transmitted from primary sensory
neurons to the neurons that ultimately mediate homeostatic responses
(i.e. to neurons that regulate behavior, endocrine responses or
autonomic output).
We
are also interested in how sensory transduction, encoding and
downstream synaptic signaling can be modulated to affect homeostatic
reflex gain at the cellular and network levels. Changes of this
type, for example, could mediate the circadian modulation of
osmoregulated vasopressin release that prevents dehydration during
sleep. The sketch on the left illustrates one approach which is used
in our laboratory to perform such studies. In this case,
osmoreceptor neurons in the OVLT are briefly exposed to an osmotic
stimulus while a whole cell patch clamp recording is made of the
synaptic response in a vasopressin releasing neuron in the
supraoptic nucleus (SON). Eric Trudel, a recent PhD student in the
Bourque Lab, used this approach to show how circadian changes in the
firing rate of clock neurons in the suprachiasmatic nucleus (SCN)
can facilitate the osmotic activation of SON neurons during late
sleep. See comments on this work in
Scientific American and
Science News Magazines.
Regulation and function of firing patterns. We are
interested in how the expression and neuromodulation of ion channels
can shape electrical patterning in osmoregulatory neurons. We are
also interested in defining the significance of different
patterns in neural communication. For example, action
potential
bursts can alter the strength of synapses between OVLT and SON
neurons and therefore provide a mechanism for gain control in this
pathway that may be
relevant under specific physiological conditions. Another example is
the phasic bursting activity pattern that is adopted by vasopressin
releasing neurons during haemorrhage or dehydration (right). This
pattern is known to optimize peptide release from the neurosecretory
endings of these cells. Orevious work by Colin Brown in the Bourque
Lab has shown that activity-dependent release of dynorphin by the
dendrites of these cells plays a key role in the cell-autonomous
control of firing pattern.